Another week, another element. Let’s head off on another exciting elementary excursion. You’ll get the idea now that I hope to ensure that at least one element is included from each of the eighteen Groups of the Periodic Table (a.k.a. the Periodic Table of the Elements).
So far, we’ve taken a trot through the stories of elements from nine of these:
- Group 1: Hydrogen, and Sodium
- Group 2: Calcium
- Group 8: Iron
- Group 10: Platinum
- Group 11: Copper
- Group 12: Mercury
- Group 13: Boron
- Group 14: Carbon
- Group 16: Oxygen, and Sulphur
- Group 17: Bromine
On this occasion, we’re going to take a look at an element which surprised me right out of the starting gate. This is the one which, being the lightest of its stablemates, tops the charts for Group 4, the metallic element titanium.
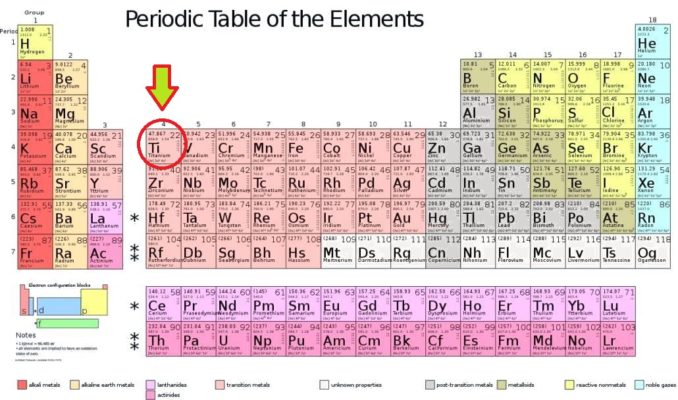
2012rc, licensed under CC BY 3.0
Why the surprise? Well, to me it has always sounded a pretty innocuous metal, after all, it is biocompatible—medical grade titanium is used in implants and in reconstructing broken bits of the body. What I hadn’t really thought through, indeed wasn’t really aware of at all, was its reactivity.
I must admit that I had rather anticipated that this abundant element (one making up c. 0.56% of the Earth’s crust) would be found in native form, even if it was more commonly found as an ore (in compound form). Nope. It is by no means the nicely behaved inert stuff I had thought it was.
OK, we are not looking at the same level of reactivity the likes of the alkali metals exhibit (Group 1 on the Periodic Table), but titanium is still a very reactive metal. Titanium metal (when hot) even reacts with the normally unreactive gas nitrogen. As the reaction is so exothermic, titanium is the only element to ‘burn’ when exposed to pure nitrogen gas.
Interestingly, this reactivity is disguised, to some degree, as titanium (and its Group 4 associates) all readily form a dense and compact passive oxide layer which binds tightly to the surface of the metal. The oxide layer (in titanium’s case, titanium dioxide, TiO2), not only protects the metals from further corrosion, reactions with atmospheric oxygen, but it also limits accessibility to the pure metal for other agents. This means that their ability to react with water, and many acids and alkalis, is greatly reduced. It is this layer which had me fooled about our element’s reactivity!
That thin layer of oxide provides excellent corrosion resistance, protection so good that it’s said that after 4,000 years in seawater, corrosion ingress would be ≤ 1mm. Titanium can, however, despite its resistance to corrosion, be attacked by very concentrated acids, such as hydrofluoric acid (HF), so it is possible to etch it.
Titanium, which has been assigned the symbol Ti, is the 22nd element of the periodic table, a d block transition metal, in Group 4, Period 4. Our element is believed to be produced by stellar nucleosynthesis, that is when stars (typically massive stars or white dwarfs) explode, lighter elements fuse together into heavier ones.
On Earth, titanium is most commonly found in minerals such as rutile (titanium oxide, TiO2), ilmenite (iron titanium oxide FeTiO3) and sphene, a.k.a. titanite (calcium titanium silicate, CaTiSiO5). The pure metal is silvery-white to silvery-grey in colour (just a little darker than aluminium).
In elemental form, it is lightweight, strong, hard, and lustrously shiny, and is classed as a ‘refractory’ metal. This means that it has excellent resistance to heat (these metals have a melting point higher than 2,123 Kelvin, 1,850 °C). It’s ductile and malleable, particularly when heated. It has a high strength-to-density ratio (in fact, the highest of any metallic element), making it very wear resistant. It can be alloyed with a number of metals, such as steel (which is quite common) but also aluminium, copper, iron, manganese, molybdenum, nickel, palladium, and vanadium, extending its use yet further.
Our element is unlike many other metals, as it isn’t a good conductor of heat and electricity. Titanium usually donates electrons to form compounds, with most common oxidation states of +3 and +4. Simple, eh? Not quite so straightforward, because most transition metals have multiple oxidation states. Titanium can actually form compounds with the following oxidation states: −2, −1, 0, +1, and +2, as well as +3 and +4.
Titanium has five stable isotopes: 46Ti, 47Ti, 48Ti, 49Ti and 50Ti. Of these, 48Ti is the most abundant (at c. 73.8%). Nuclear physics research uses 48Ti, and it is used to produce the vanadium radioisotope 48V, used in nutritional studies and in cancer imaging. Meanwhile, 46Ti is used in neutron activation detectors to determine the concentrations of elements in materials, 47Ti is used in analytical techniques to examine irregularities in the structure of crystals, glass, and ceramics, and 50Ti is used to synthesise super heavy elements, bombarding either lead or bismuth.
There are a further twenty-one radioisotopes, with the most stable, 44Ti, having a half-life of 60 years. To date, none have found applications.
Titanium has two main allotropes.
- alpha titanium (α-titanium), with a hexagonal close-packed (HCP) lattice – easily welded, it’s not the strongest but is still relatively tough, even at cryogenic temperatures. It has the best machinability, and the best corrosion resistance of the allotropes.
- beta titanium (β-titanium), with a body-centred cubic structure – is the most durable, relatively pliable, has higher tensile strength, and can withstand extreme heat. In fact, this allotope is formed at temperatures greater than 882 °C.
But titanium is also found as a mix of these allotropes. In effect, this is categorised as a third allotrope, called alpha-beta titanium (α+β titanium). This has a range of properties, combining those of alpha and beta (for example, it is structurally stable, tough, exhibits decent plasticity, and high-temperature resistance to deformation) which makes it particularly valuable to industries which use titanium, particularly when alloyed with other metals.
Titanium’s discovery is an interesting tale. It was first isolated as an element in the late 1700s, being recognised as an ‘unknown element’ by a young Cornishman, Reverend William Gregor, a keen mineralogist known as the ‘Scientific Parson’. He was the rector at the church of St Crida (between Truro and St Austell), but often called in on his friend, fellow clergyman, Reverend Richard Polwhele, at his church in Manaccan.
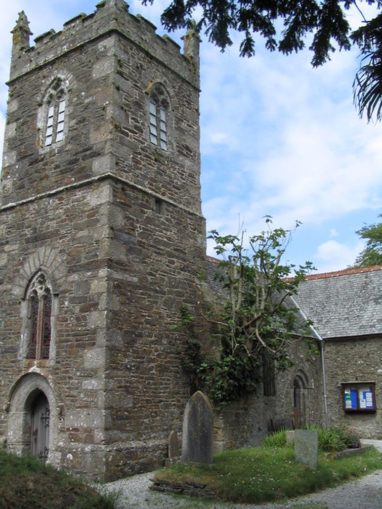
Tim Heaton, licensed under CC BY-SA 2.0
Whilst visiting, either in 1790 or 1791 (accounts vary), Gregor collected samples of a black sand, weathered out of the local gabbro rock, from the nearby Tregonwell Mill on Gillan Creek to analyse. Though he didn’t know it, this sand was a titanium-iron oxide known as ilmenite. This mineral is actually titanium’s most important commercial ore, worldwide, and now accounts for around 92% of all titanium extracted. Gregor, however, was intrigued by this material as it ‘followed the compass’, that is it was mildly magnetic.
His analyses revealed that the sand contained iron (the source of its magnetism), manganese, and another substance which he successfully extracted (as a reddish brown ‘calx’) but could not identify. He called this new substance ‘manaccanite’ (sometimes written as ‘menachanite’, ‘menachite’, or ‘menachine’), honouring the parish in which it was found. He reported his exciting discovery to the Royal Geological Society of Cornwall. There is a nice plaque commemorating titanium’s discovery at the church at Manaccan.
Being a diligent scientist, Gregor also published his findings in the German scientific journal ‘Crell’s Annalen’ and the French journal ‘Observations et Mémoires sur la Physique’.
But if ‘manaccanite’ is what Gregor named it, where did the name titanium come from?
Well, a few years later, in 1795, renowned German chemist Martin Heinrich Klaproth (who also who discovered the element uranium) was analysing a sample of the ore rutile, also known as ‘red schorl’, from Hungary. He was delighted to realise that he had unearthed a new element, and proposed that this new element be called ‘titanium’ after the pre-Olympian gods, the Titans, of Greek mythology, writing:
“Whenever no name can be found for a new fossil which indicates its peculiar and characteristic properties (in which situation I find myself at present) I think it best to choose such a denomination as means nothing of itself, and thus can give no rise to any erroneous ideas. (as Lavoisier had suggested) In consequence of this, as I did in the case of Uranium, I shall borrow the name for this metallic substance from mythology, and in particular from the Titans, the first sons of the earth. I therefore call this new metallic genus Titanium.”
A measure of the scientific ethics of the man, when Klaproth became aware of the work Gregor had previously published, he obtained a sample of the black sand from Cornwall to analyse. He compared the results with those from his Hungarian material. He duly confirmed that the element in the red oxide ‘calx’ (albeit derived from two different minerals) was identical. He graciously assigned the credit for titanium’s discovery to Gregor.
Would that happen nowadays? I’ll let you decide about that. However, the name Klaproth chose stuck, and we call it titanium to this day.
Now that a new element was recognised, Klaproth and others began the painstaking process of obtaining a pure sample. Many tried, but this was anything but easy. It took almost another hundred and twenty years. Extracting titanium from its various ores is long, laborious, complicated, and costly.
Ultimately, in 1910, the New Zealand metallurgist, Matthew Hunter, succeeded, producing an extremely pure (99.9% purity) specimen. Nevertheless, his work modified a process previously exploited by Lars Nilson and Otto Pettersson, in 1887, who’d managed to extract titanium with a purity of c. 95%.
Hunter combined titanium tetrachloride (TiCl4) with sodium (Na) in a ‘metal bomb’, an airtight steel cylinder, then heated this to 1,000 °C. This became known as the Hunter process.
This really wasn’t the safest process. Sodium isn’t terribly user friendly. Add this to the high temperatures and pressures required, and it isn’t too surprising that Hunter chose to conduct many of his experiments on the football field of his New York institution! However, his method prevented newly extracted titanium from reacting with atmospheric nitrogen or oxygen, to obtain high purity titanium.
Unfortunately, Hunter’s process was fairly inefficient, with much effort yielding a relatively small amount of pure metal. That made titanium principally a laboratory curiosity. Nonetheless, his methodology is still used where particularly pure titanium is required.
This was superseded, commercially, in 1940 by the much more efficient Kroll process, developed in Luxembourg by metallurgist William Justin Kroll. With this improved technique, TiCl4 is reduced by liquid magnesium (he’d originally used calcium) to extract the titanium metal. Still a complex, time-consuming, energy intensive, multi-step process, it remained extremely costly to produce titanium, despite the yields being greater.
Very briefly summing up Kroll’s method, ilmenite (FeTiO3) or rutile (TiO2) ore is heated with carbon to about 1,000 °C. Then chlorine gas is passed over the mix, producing titanium tetrachloride, TiCl4, nicknamed ‘tickle’. Then, under a blanket of inert argon gas, the ‘tickle’ reacts with liquid magnesium, at 850 °C (the magnesium is liquid as this is above its melting point). This gives you the ‘titanium sponge’.
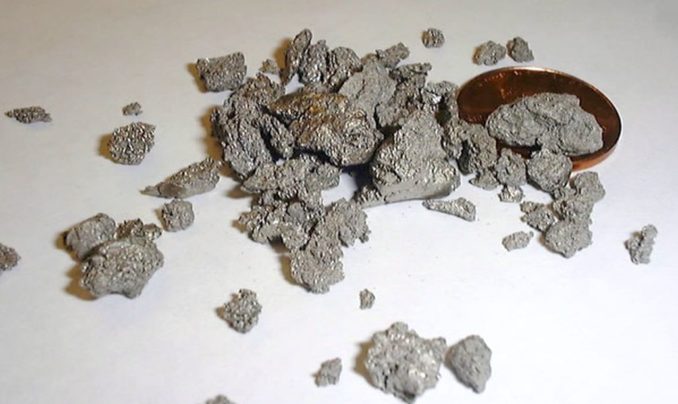
US Government, Public Domain
The porous titanium sponge then needs to be purified, which can be achieved through vacuum distillation. Even then, the titanium sponge needs to be compacted before being melted to form an ingot. This melt requires a vacuum arc furnace. The vacuum needs to be maintained so that the ingot can solidify without reacting with atmospheric gases. As you can see, it isn’t an easy process. In truth, things are slightly more complex than this, but that’s the bare bones.
Despite Kroll’s great step forward, nearly a decade on, global production of our metal only stood at a meagre 3 tons a year (figures from 1948). It didn’t help that, at that point, titanium was still categorised as a ‘brittle’ metal. This isn’t too surprising since when the ore is processed, chemically reducing the mineral to extract the metal, it is produced as a highly porous, brittle mass (the ‘titanium sponge’). This doesn’t look much and, together with this slightly denigrating description, meant that it was mainly used in alloys, or to deoxidise steel.
Conversely, our metal’s importance, and the multiple uses to which it is put nowadays, is clearly evidenced by current figures for titanium production, worldwide. In 2022, global production was some 9.49 million tons. Not bad, since the still exorbitant production costs mean that titanium costs about nine times as much to produce as stainless steel.
Potential applications for titanium remain an area of considerable interest. Currently, the largest titanium mineral reserves (in ore form, typically as ilmenite, but also as rutile) can be found in China, followed by Australia, then India. There are also significant deposits in Brazil, Canada, Japan, Mozambique, Norway, Russia, South Africa, and other countries, including Ukraine. Titanium production (extraction from its ores) today is also dominated by China.
OK, we now know a bit about where titanium comes from, but what is it used for?
It may come as no great surprise that military applications were the first to be identified, with the Cold War the main driver. Titanium’s light weight, high strength to weight ratio, resistance to corrosion and temperature (managing thermo-elastic stress at extremes of both heat and cold) made it an appealing material for a variety of military applications.
The Soviet Union were the pioneers in its use (at that point they also held the largest known reserves). Although they had previously used it in steel alloys, considerable research into using titanium in its own right got under way in the early 1950s. Large-scale industrial production was set up across the USSR, in Ukraine (near Mariupol), the Urals, and Kazakhstan.
From the late 1950s onwards, the Soviets increasingly trialled titanium in their aircraft design. Indeed, the faster an aeroplane is designed to fly, the greater the friction (which produces a lot of friction heat) so the higher its anticipated requirements for titanium. That said, it is still not quite clear when, or with which of their aircraft, titanium usage actually began.
However, their progress greatly worried the Americans. US concern is evidenced by an article from 1956 in ‘American Metal Market’ which exclaimed “Soviet Output of Titanium May Be Greater Than Ours“. This resulted in some terse correspondence between the CIA and the United States Senate. As an aside, given the war in Ukraine, titanium supply is still a matter of some concern now, as this 2022 brief indicates.
However, the Soviet’s use of titanium to the extent the Americans feared was not necessarily true. They’d expressed concerns that the Mikoyan-Gurevich MiG-25 ‘Foxbat’ (nominally capable of Mach 3.2+ flight speeds) was titanium-built. This aircraft had set a world speed record in 1967, achieving 2,981 kph (in theory, it could fly at Mach 3), and altitude records in 1973, attaining 118,900 feet.
The MiG-25 was viewed as a significant threat to NATO aircraft at the time. Robert Channing Seamans Jr., US Secretary of the Air Force, said of the ‘Foxbat’ that it was: “probably the best interceptor in production in the world today.”
However, although some titanium had indeed been used in heat-critical areas, the fact that titanium is so difficult to work with meant that the MiG-25 was for the most part constructed (mostly hand welded at that) of nickel-steel, a.k.a. stainless steel.
NATO was unaware of this, and that reassuring nugget of information was only finally confirmed when Lieutenant Viktor Belenko defected in September 1976. He was the Russian pilot who, in the process of getting out of the USSR made off with a ‘Foxbat’, landing it in Hokkaido. This allowed Western military intelligence not only to get a close look at the plane itself, but Belenko also brought news of its successor, the Mig-31 series.
In the meanwhile, the US military was busily playing catch up. In a race against time, they tried to steal a march on the enemy by developing their own air capability. There was a small hitch though, since the majority of available titanium came from the world’s major producer… the Soviet Union!
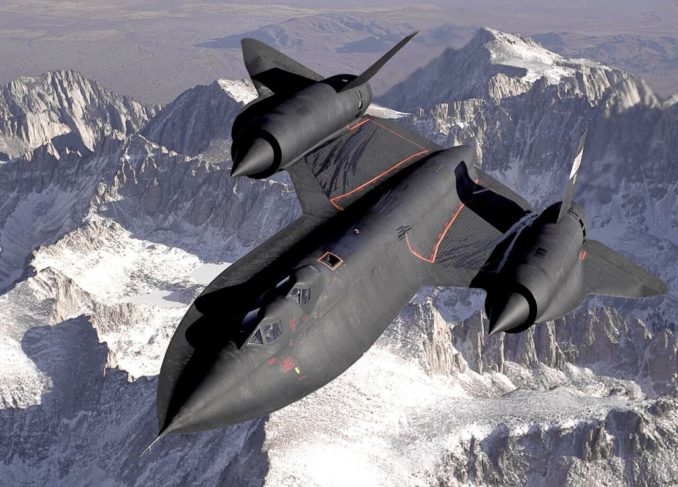
USAF, Public Domain
The US did have some reserves of the required ores, but precious little for what they had in mind, a fleet of high-altitude, long-range planes with Mach 3+ capabilities (for strategic reconnaissance, as interceptors and as drone-launchers). They were going to need a lot!
No problem, the CIA, sneaky as ever, got to work. They used a variety of third parties, ghost organisations, and dummy companies, to quietly acquire the required metal from the Soviets. Believe it or not, they even persuaded the Soviets that the American public were too darn lazy to cook for themselves and were partial to pizza, so the metal was needed to produce thousands of pizza ovens!
Titanium was fundamental to the eventual production of Lockheed’s thirteen A-12s, two M-21 drone carriers, and three YF-12 fighters. More importantly, it was crucial to Lockheed’s thirty-two ‘spy in the sky’ SR-71 ‘Blackbirds’. The Blackbird was a mighty beast, significantly larger than the A-12, and was 92% titanium, inside and out.
Even getting hold of the requisite titanium, however, wasn’t enough. As the Soviets had discovered, developing technologies to overcome the difficulties of working with this ‘wonder metal’ was a feat in itself. This took place at Lockheed’s ‘Skunk Works’, and is worthy of an article of its own—thankfully, descriptions of some of the problems and the solutions to them are laid out in a really nice article ‘Titanium and the SR-71’.
Military uses aside though, money talks, and with a strident voice. The first commercial application for titanium came in the early 1950s, also in the aircraft industry. The American aircraft manufacturer, the Douglas Aircraft Company, introduced a new airliner, the Douglas DC-7, first taken into service by American Airlines in 1953.
At the time it was hailed as the world’s fastest airliner (with a top speed of 410 mph). This was, perhaps, slightly surprising as the DC-7 was the last of the propeller planes Douglas produced. It hit the skies soon after the first commercial jet airliner (the British built de Havilland Comet) went into service.
But the DC-7, which had a range of over 3,000 miles, had a bit of a secret weapon. The construction incorporated titanium, which was used initially for the nacelles and firewalls. Use of our metal provided a weight saving of around 200 pounds per plane for Douglas (titanium is as strong as steel but 45% lighter) and was one reason that the DC-7 was first to fly nonstop westbound across the USA, against the prevailing jet stream winds.
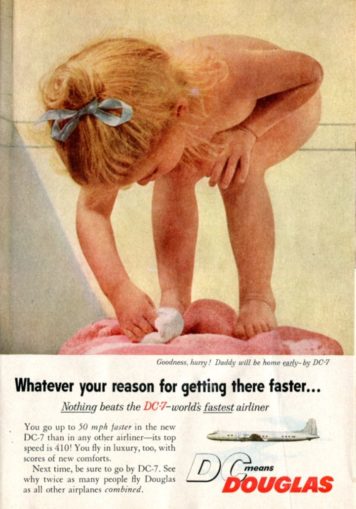
SenseiAlan, licensed under CC BY 2.0
A luxurious way to travel, the DC-7 pretty much revolutionised air travel, and the use of titanium in this plane was the start of a long association with aircraft which continues to this day. It’s still the fastest growing market for titanium and titanium alloys. Much of the titanium can be found in the jet engines, but it is also used in structural components (fuselage frames, landing gears, hydraulic lines, bulkheads, and skins).
For example, today’s Airbus A380 (the largest airliner ever built) features over 70 tons of titanium, mostly in the form of Grade 5 titanium alloy (the alpha-beta titanium alloy Ti-6Al-4V), in its construction. This represents around a quarter of its operational empty weight. Its main rival, the Boeing 747 incorporates c.45 tons of titanium.
Interest in this ‘wonder metal’ soon extended to the wider aerospace industry. Today, around two-thirds of all titanium produced worldwide is used by the industry. Even when helicopters, planes, and spacecraft are still at the design stage, minimising load is critical. The biggest challenge is to optimise the weight of components without compromising strength or performance. Titanium and its alloys are amongst many materials (we considered boron and its composites in the last article) which are crucial to this.
Even components which we’d not necessarily consider need careful thought. A prime example is an upgraded toilet design, installed on the International Space Station a few years ago, replacing the clunky thirty-year-old Russian model. Costing just a snip, at $23 million, the titanium Universal Waste Management System (UWMS) will allow astronauts, particularly those of the female variety, to ‘boldly go’ for many years to come.
But it’s not just above ground where titanium made its mark. Dominance in the air was only one aim of the Cold War combatants. This aspect of the arms race bothered the Soviets, as for the first couple of decades, their naval fleet was dependent upon outdated technology. This included the diesel-electric attack ‘Whiskey’ (and nuclear-powered ‘Echo’) class submarines, whose technology derived from designs captured from the Germans at the end of WWII.
So, through the 1950s and 60s, the Soviets placed considerable importance upon submarines. This resulted in a long period of ‘forced’ development for techniques to work with titanium. A raft of mistakes and problems were overcome along the way until, on completion, the K-222 (originally known as the K-162), the first and only Project 661, Papa class sub was launched as the first ever titanium-hulled submarine.
She was specifically designed (from the bottom up) as a highly innovative craft to neutralise the threat from American aircraft carriers. Her engineers and designers had been expressly prohibited from re-employing earlier designs and technical solutions.
Commissioned in 1969, she was the world’s fastest submarine, capable of a tremendous 44.7 knots (51.4 mph, 82.78 kph), setting an underwater speed record that is still-unbeaten. Amazingly, this pace was achieved with the nuclear reactor operating at around 97% capacity. Theoretically, she was faster still—unofficially, she was clocked at 44.85 knots.
But K-222’s construction proved too complicated to be anything more than a prototype. She was also ridiculously expensive, with estimated costs around two billion roubles (about 1% of the USSR’s GDP in 1968), thus she earned herself the derogatory nickname ‘Golden Fish’. Nevertheless, technical solutions achieved during her construction didn’t go to waste. Many were used in later Soviet submarines.
One-off or not, she certainly put the wind up the Americans. In 1971, she tracked the USS Saratoga, as the ship was underway with the Sixth Fleet from the Mediterranean to Miami. Although the Saratoga was running under full power, K-222 outpaced her. Worse was the realisation that, had she chosen to, K-222 had multiple opportunities to attack.
Meanwhile, the Soviets were following the K-222 prototype with Project 705 Lyra, Alfa-class submarines. Designed as swift, readily deployed interceptors, these diminutive subs were capable of high speeds (≥ 41 knots), rapid acceleration, and extremely deep dives, at 2,200 feet (366.67 fathoms) deeper than the effective range of any US antisubmarine weaponry.
Alfa’s small capacity meant a limited crew size, typically just 31 people, but these were the cream of the Russian Navy. An all-officer crew was said to enhance combat effectiveness. In fact, operating with such a tiny number served to drive the automation of a number of key systems.
All this, together with further innovations made the Americans loath to believe it could be possible, even once the sub was first observed in 1969 at the Sudomekh Submarine Shipyard in Leningrad. Their rather arrogant standpoint was, if we can’t do it, how on earth could the rapscallion Russkies?
Titanium hulled subs were also difficult to detect magnetically (titanium is barely magnetic, classed as paramagnetic, so cannot be identified using magnetic anomaly detectors, MADs). The innate strength of the hull better protected the sub from depth charges. As well as the titanium construction, Alfas were powered by a compact, innovative, lead-bismuth cooled reactor. This small but powerful design minimised the overall size of the submarine, but still permitted very high speeds, making these ‘silent killers’ (which were actually quite noisy, particularly at high speed) a scary proposition.
Despite the alarm the prospect of these prowling the seas prompted, did the Americans (and NATO) rush to emulate these worrying innovations in submarine design? No. Rather sensibly, they chose instead to focus their efforts on much less costly high-speed anti-submarine weaponry, developing effective countermeasures capable of catching and destroying Alfa-class subs.
The lethal Mark 48 Torpedo was one such solution, the Royal Navy’s ‘Spearfish’ torpedo another. I can’t resist this photo, not least for the gratuitous display of ‘Snap-On’ tool chest porn.
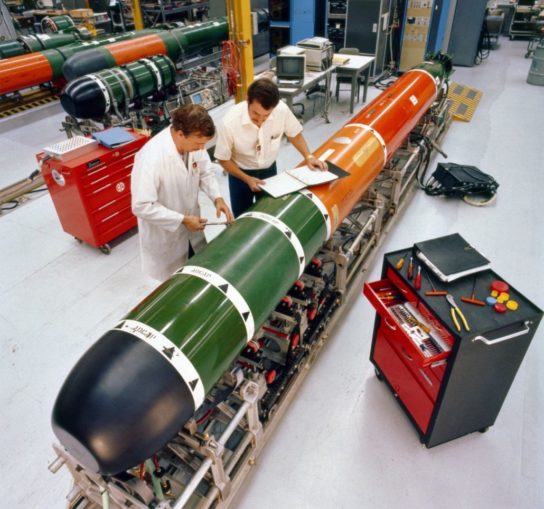
United States Navy, Public Domain
The massive cost savings achieved by not pursuing titanium-hulled subs probably gave NATO a considerable financial advantage, particularly since NATO did not have access to the abundant titanium ores which the Soviets held.
So, we’ve seen the rise of titanium in the air and under the sea, but what of on land?
US research into the potential of titanium alloys began in the 1950s, with the Watertown Arsenal leading the way. This facility later became a part of the U.S. Army Combat Capabilities Development Command Army Research Laboratory (DEVCOM ARL). There’s a fascinating report from a 1952 Symposium (accessible here: https://apps.dtic.mil › sti › tr › pdf › AD0635553.pdf). Norman L Reed’s section on ‘Titanium in Ordnance’ is interesting, but I found Francis G. Tatnall’s section on ‘Titanium As A New Products Potential’ particularly thought-provoking as it predicted potential applications outside military use.
Inspired by new materials, the revolution in aircraft technology, and the space race, General Motors got in on the act, with their ‘Firebird’ experimental ‘dream cars’. Seriously cutting edge, the first in the series showcased in 1953. Firebird I (XP-21) was the first gas turbine-powered car. Totally impractical, it was, in effect, a jet on wheels, with a bullet-shaped fibreglass fuselage.
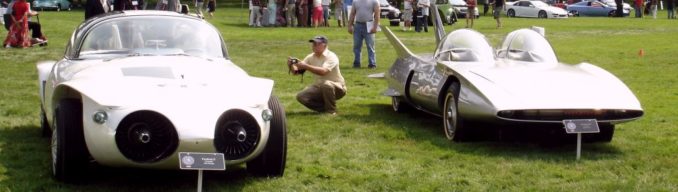
Karrmann, licensed under CC BY-SA 3.0
Next came Firebird II, in 1956, a turbo-powered four-seater sports car, this time featuring an all-titanium body. This futuristic delight features in the US Auto Industry 1956 film ‘Design for Dreaming’, popping up in all of its sci-fi splendour at 7:17. It’s a wonderfully cringe-inducing little film now—the kitchen of the future is as much fun as the car.
General Motors, sadly, reverted to steel and fibreglass for their gull-winged 1959 Firebird III. I guess they realised that titanium was always going to be prohibitively expensive for the bodywork of production cars, but it’s strength means it is still used for the structural frameworks of some racing cars, as well as racing karts, and motorbikes (like Clews’ CCM special edition Spitfire and their Heritage ‘71 Ti).
But the concept of titanium bodies didn’t completely die out. An Italian company, the Icona Design Group, built a one-off collector’s supercar in 2016, inspired by the iconic Blackbird SR-71. With its supercharged 6.2-litre V8 engine, this baby was fast. It boasted a top speed of 220 mph, plus incredible acceleration. I have no idea what has happened to this beast in the intervening years, but had you wanted it when it was first built Icona’s Vulcano Titanium could have been yours for a mere £2 million.
But it isn’t only automotive horsepower that had the titanium treatment. Being very ductile and malleable, titanium (or rather, its alloys) is one of relatively few metals that can be used for 3D printing. As we’ve already seen, other properties, such as lightness, chemical resistance, strength, thermal characteristics, even biocompatibility, make it an attractive substance for a range of applications.
One of these is to make horseshoes. Yes, you read that correctly, shoes… for horses. Titanium shoes weighing much less than traditional steel shoes. Furthermore, they can be created to be half the weight of an aluminium racing shoe. The lighter weight, plus better flexing properties, reduces strain and fatigue which can impact both a horse’s performance and its hoof health.
The obvious use for these lightweight shoes is in horseracing, potentially giving a horse an advantage over its rivals. Australian trainer, John Moloney, collaborated with Australia’s national science agency, the Commonwealth Scientific and Industrial Research Organisation (CSIRO) to fit one of his horses, rechristened ‘Titanium Prints’, with the high-tech shoes. Was the horse a dead cert in its next race? We’ll never know, but the cost of these shoes is probably the reason they don’t appear to have been adopted more widely in racing.
However, 3D printing (using advanced electron beam melting, EBM, technology) allows ‘perfectly’ fitting custom shoes for each hoof to be made, in an equine equivalent of orthotics. The shoes can, if necessary, be glued into place, rather than nailed on. Both factors can be really beneficial for horses with diseases of the feet, for example laminitis (an extremely painful condition), allowing them to walk out comfortably when they have sometimes suffered for years. There’s a nice video of Holly the Pony happily trialling her new CSIRO shoes. The video explains the process from initial scanning of each hoof to fitting within only a few hours.
The same properties, especially the strength for light weight, make titanium (or titanium alloys) a good choice for a range of high-end sports equipment. Things like golf clubs, tennis rackets, bicycle frames, mountain and rock-climbing equipment, fishing rods and tackle, fencing equipment (including blade guards and protective masks), and my personal favourite, diving knives. Such equipment tends to last longer than that made from traditional materials, not least because it’s really rather hard to damage it.
These very properties, e.g. weight, strength, and overall chemical resistance, plus the ability to maintain a good cutting edge, also make titanium a good choice for surgical instruments. In addition, since it has such a high melting point, autoclaving the instruments to sterilise them poses no problems at all.
Probably a better bet that these nicely corroded Graeco-Roman surgical instruments, eh. Don’t even ask what the one on the right is for!
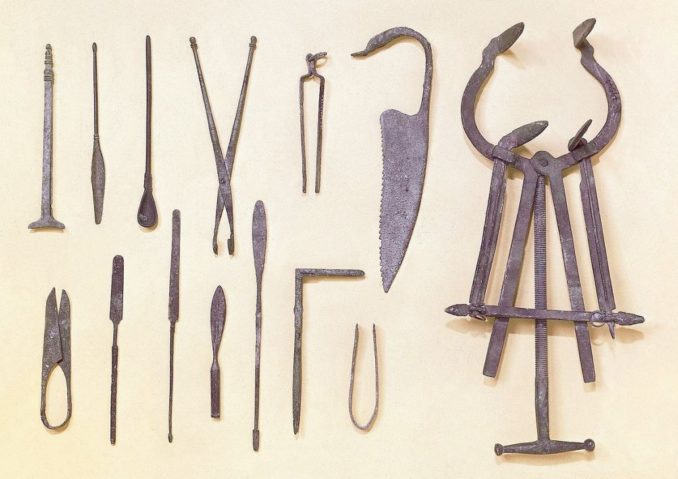
Wellcome, licensed under CC BY 4.0
Mention was made of titanium’s biocompatibility too (it is non-toxic, non-allergenic, and is not rejected by the human body, although it plays no role in human metabolism). Both its density and elasticity are comparable to that of human bone. Better still, it can to some extent ‘fuse’ with bone tissue in a process known as osseointegration.
This makes titanium (in the form of Grade 23 titanium alloy, Ti-6Al-4V-ELI) a valuable material for pins, plates, rods, meshes and cages used in reconstructive surgery, as well as the screws that hold them in place. Titanium also finds a use in cochlear implants to restore hearing, and in artificial joints, e.g. for hip or knee replacements.
It’s used in dentistry too, in braces to realign teeth, as a supportive structure for implants, bridges, crowns, and even partial or full dentures. A slightly less critical, semi-medical application is for spectacle frames. Here the strength and light weight are key, but being hypoallergenic also helps.
There are also cardiac and cardiovascular applications, from stents to replacement heart valves. One of its alloys, nitinol (55% nickel and 45% titanium) possesses thermal shape memory properties, so can be ‘folded’ for insertion through a tiny aperture, then released to open out to the required shape and size. This is ideal for inserting self-expanding stents.
Oh, and implanted pacemakers need protective cases too, so titanium finds a place here. Being strong, and inert is great, but also being non-magnetic means that implanted titanium will even allow a patient to undergo subsequent MRI scans without causing a problem.
Titanium alloys have found a number of uses in electronics, particularly where the environment is hostile (e.g. connectors, switches, and battery terminals in marine electronics where you really don’t want things to corrode). Other relatively rugged equipment, such as Apple’s PowerBook laptop bodies, also use titanium.
Titanium and its alloys are used by the chemical and petrochemical industries too, in tanks, valves, pipes, and batch process vessels (particularly pressurised vessels). Likewise, the pulp and paper industry use titanium alloys, since the corrosive materials used in paper production require any equipment to possess excellent corrosion resistance.
Before I move on, it is worth saying that titanium has found some uses in aesthetic projects, including in both sculpture and architecture.
At Leninsky Prospekt in Moscow stands a massive, ribbed metal column topped by a figurine, in total, nearly one hundred and forty feet tall. The metal is, unsurprisingly, titanium, and this striking piece is the world’s first large-scale monument to have been made with this extraordinary metal.
The sculpture was created for Moscow’s 1980 Summer Olympic Games, by Soviet artist Pavel Ivanovich Bondarenko. It was erected to honour the first human being to journey in space, and the first to orbit the planet Earth.
In April 1961, aboard the Vostok 1, the man who briefly ruled the world was the Soviet pilot and cosmonaut Yuri Alekseyevich Gagarin. It may be sacrilege but, if I’m honest, I think his statue is decidedly ugly and does no justice at all to this brave young man. I feel that the one at the Royal Observatory in Greenwich, whilst zinc not titanium, is infinitely superior.
Perhaps the best-known example use of titanium in architecture is the cladding on Canadian American architect Frank Gehry’s Guggenheim Museum Bilbao. The museum, in northern Spain, opened in 1997. Set alongside the undulating River Nervión, its fluid appearance curvaceously changes, shifting with the light gently reflecting from slightly dimpled scales of titanium set at crazy angles. As the sun goes down and the light reddens, it is simply spectacular.
To say it’s an outrageous piece of architecture is putting it mildly, and I daresay the builders had a few uneasy moments trying to make sense of the plans, let alone working with the titanium. I’m not sure what Gehry was smoking, but it’s a stunning concept and one I’d love to see for myself. It actually seems to work somehow.
It is anything but mundane. Viewed from above it resembles a flower unfurling. Approached from the centre of the city towards the Salbeko Zubia Bridge, which it insinuates itself below, it rises like a battleship under full power steaming upriver, with the rippled hills in the background. Looking upwards from the river, or the opposite bank, it has the presence of a futuristic sci-fi castle.
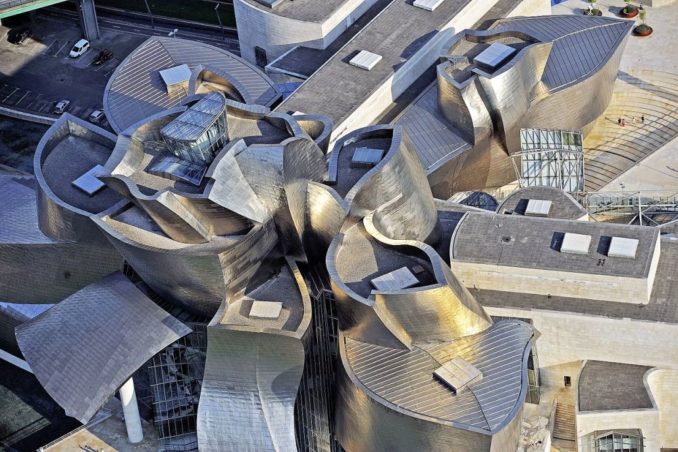
EJ-Irekia, licensed under CC BY 3.0
The slightly overlapping titanium plates or scales are actually some thirty-three thousand in number, covering 25.221 m2 of the building. The titanium is thin, only c. 0.4mm, and because titanium is a lightweight material, the cladding over the entirety of this huge edifice only weighs something like 60 tons.
Amazingly, this work of architectural art was completed on time and within budget (HS2, eat your heart out). Funded by the Basque government, OK, the Basque people, in the first four years after opening it brought in sufficient revenue to completely cover the cost of construction. After that, the money it brings to the city is pure profit.
An interesting point, and one which I’ve been unable to properly confirm, is whether some of the titanium used in this incredible museum (which is known to have been sourced in Russia) might just have come from the decommissioning of old Soviet Alfa-class submarines. There was certainly a surplus of titanium readily available at the time, dropping the price per ton. What’s more the beginnings of the museum’s construction phase happened to coincide with five of the vessels being broken for scrap in 1990. Could this cladding be a Cold War remnant? Who knows.
Well, the metal and its alloys have certainly found some interesting applications, but as we saw at the beginning of this piece, titanium is an element which is happy to combine with other elements in compound form. So, what about the uses to which these have been put?
Titanium atoms will happily bond directly with carbon atoms, so organic compounds can be formed. There has long been significant interest in these, and there is a sizeable branch of chemistry dealing with this very complex topic, but the first compound isolated and characterised which contained Ti—C bonds was only accomplished in the early 1950s. These types of compounds, which are actually known as organotitanium compounds, are primarily used as reagents in industrial processes, often as catalysts or in the synthesis of other materials.
The most important compound, in commercial terms, is titanium dioxide, TiO2 (a.k.a. titanium (IV) oxide). In fact, the use of this compound alone comprises around 95% of all titanium used worldwide.
Titanium dioxide is a chemically stable, unreactive compound. It is naturally occurring as a mineral, in fact as a couple of minerals (primarily as rutile and anatase). However, as so much of it is used for a variety of different applications, it is also produced from titanium’s ores (here, primarily from ilmenite), using methods similar to those we’ve already seen used to extract the elemental metal. Once produced in this way, it is a fine white powder.
It’s an extraordinarily versatile compound. One of its main uses is as a brilliant white, non-toxic pigment, in fact the whitest and brightest pigment there is, so think of titanium the next time you dip a brush into a pot of Dulux.
It’s actually found in around 90% of paint products, both domestic and industrial (it replaced white lead when lead paints were banned in the UK in 1992). Its properties help to protect the surfaces to which it is applied, and it is the white pigment used in the ‘white lines’ on our roads, so helps protect us too.
It isn’t only for a nice bright white; you’ll find it in coloured paints too, where it helps to make the colour appear even and strong. As it is excellent at reflecting and scattering light, it is even used to make glittery, iridescent and or pearlescent type paints. It also acts as an excellent opacifier (helping to hide whatever is already on the surface to be painted) and has a very high refractive index, so reflects visible light well.
But it’s not just used in decorating paints, but art paints too (acrylics, oils, pastels, and watercolours), where you probably won’t be surprised to hear that it is very often called ‘titanium white’. It’s even in tattoo pigments.
Titanium dioxide is also used in plastics, to make them opaque or to allow colours to be added to them. It improves their resistance to heat and light (especially ultraviolet radiation which ages many plastics, making them brittle and prone to failure), thus extending their useful life. This helps improve their weather resistance too, which is valuable in construction where PVC doors and window frames are now commonplace. It can also modify the mechanical properties of plastic products, making them easier to process. The plastics industry is actually the second largest user of titanium dioxide.
That opacifying property means that titanium dioxide has also found a major application in paper manufacturing. It not only gives a good whiteness, but because of its strong ‘hiding’ power you don’t see the print on the sheet beneath the one you are reading. It also provides a slight sheen to the paper and improves its resistance to ultraviolet rays. Papermaking, particularly for the better-quality papers, is the third largest user of this titanium compound.
Titanium dioxide is also found in cosmetics, from soaps and skin care products, to make-up, especially in foundation powders, eye shadows and lipsticks. Remember those glittery paints that scatter visible light? This property is also exploited in eye shadows.
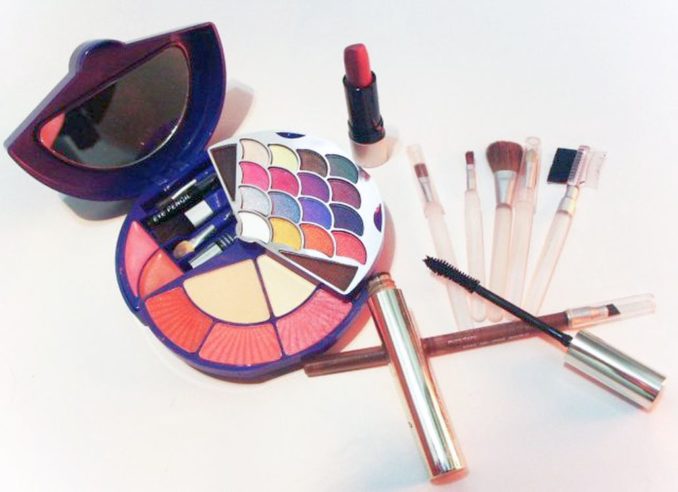
KaurJmeb, licensed under CC BY-SA 3.0
Moreover, it is an important component of sunscreen, a.k.a. sunblock. In the so-called ‘mineral’ (as opposed to chemical) sunscreens, tiny particles of titanium dioxide remain on the surface of your skin (the mineral is not absorbed) to form a physical barrier. The mineral scatters and absorbs the sun’s short-wave UVA and UVB radiation, protecting your skin from burning. It is usually combined with zinc oxide, which helps protect your skin from the longer wavelengths too.
Let’s not overlook the pharmaceutical industry, which also makes extensive use of TiO2. It’s used in a huge number of medicines currently on the market, for a number of reasons. Applications include use as a bulking agent, as TiO2 is an inert material which does not react with the active ingredients in medication. It’s also used in the protective coatings on medication, both making pills and capsules easier to take and extending their shelf-life. Adding colour to drugs is another application, especially when in capsule form, helping identify them.
Titanium dioxide is used to manufacture toothpaste, where it is listed amongst the ingredients as ‘colour index number: CI 77891’. Its high refractive index scatters visible light, so gives the paste a brightly white look, hinting at the colour your teeth ‘might’ just become once you use this marvellously marketed product.
It is even used in foodstuffs. Here it listed as the food colourant E171. As with so many other applications, it whitens and brightens the foods it is used in, making them appear more attractive to eat. It’s found in sweets and confectionary (chewing gum is a prime example), and bakery products, including bread, pastries, cakes, and breakfast cereals.
It’s in coffee creamers, ‘flavoured’ milks, and ice cream, and also in sauces and salad dressings. It isn’t only a whitening agent though, it is also used as a drying agent, and as an anti-caking agent.
In the last decade or so, there have been some major concerns about its safety in comestibles, but in June 2023 a group of ‘leading toxicologists’ from around the globe have told us it’s perfectly safe. Right, that’s the science settled then. Fancy a bit of wedding cake icing, anyone?
Titanium dioxide has a further application, which has generated quite a lot of interest in recent years. This involves its ‘photocatalytic’ properties, whereby nitrogen oxides (produced by both diesel- and petrol-engines) can be removed from the air, reducing pollution in built up areas. TiO2 is already used inside some catalytic converters, where it works with a catalyst, but without the additional assistance of light.
Photocatalysis is basically a process where a catalyst (here a specific form, or polymorph, of TiO2) speeds up a chemical reaction. The reaction is one in which molecules of nitrogen oxide are broken down into smaller units through the absorption of light. This is known as photolysis.
By painting a building with this type of titanium dioxide (or using it in another manner where it can come into contact with the pollutant) nitrogen oxides can be converted into soluble nitrate salts. When it rains, these harmless salts are then naturally washed off the surface.
Car manufacturer Toyota have already trialled this in the form of advertising billboards which were erected in in Los Angeles and San Francisco a few years back. The billboards have a titanium dioxide coating on the vinyl they are made from.
The Torre de Especialidades hospital in Mexico City has installed a100-yard-long openwork façade made of a material (a specialised cement, called TioCem) which incorporates this ‘air-scrubbing’ substance into its surface.
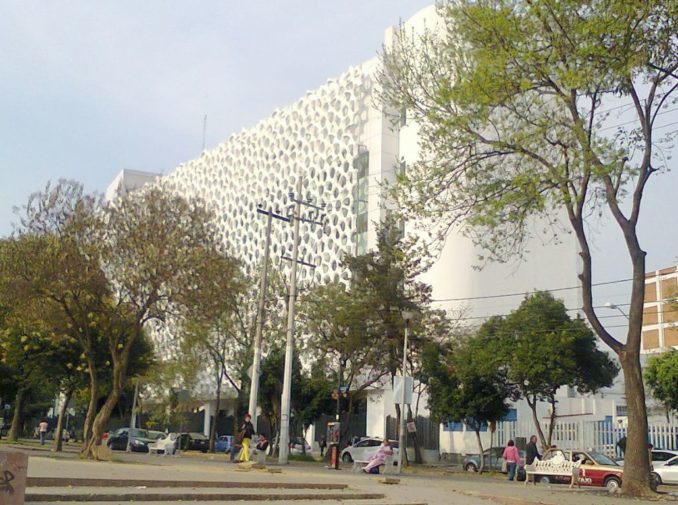
ArCaRa, licensed under CC BY 3.0
The best bit is that the TiO2 is neither consumed nor degraded in this reaction. It is simply present as a catalyst. This means that the TiO2-based coatings or materials will continue to remove pollutants from the air, day after day.
There was some talk of testing pavements and road surfaces with a layer of TiO2, as it could conceivably help remove general dirt, as well as some air pollutants from vehicle exhaust. However, I suspect that this would be a costly prospect, the wear from tyres could be a problem to overcome, and the deposition of rubber from the tyres onto the road surface may also affect long-term performance. As yet it’s probably still seen as a bit of a gimmick, but perhaps it may hold some promise. Only time will tell whether there’s an appetite for titanium dioxide’s photocatalytic properties more widely.
Interestingly, self-cleaning glass (such as Pilkington’s ‘Activ’ glass) also uses a layer of TiO2, this time very fine so it is transparent. The titanium dioxide bonds tightly to the surface of the glass, forming a hydrophilic layer which has a strong affinity for water. This means that any water spreads out and ‘sheets’ away rather than collecting into droplets to run down the glass. As it does this, dirt is carried away with the water, theoretically leaving a streak-free surface. In addition, because the layer is TiO2, the same photocatalysis we’ve seen above is in action. This breaks down some of the pollutants absorbed onto the glass surface into soluble compounds to be washed away.
OK, this is one of the compounds titanium can form. Are there others that have useful applications? Well, yes.
Possibly one of the most unusual applications I have found for titanium compounds is ‘skywriting’. This is the process by which a small plane flies in a defined pattern, typically at an altitude of approximately 10,000 feet (3 km), whilst expelling a form of ‘smoke’ to produce letters and whole words which can be read from the ground below.
It turns out that titanium tetrachloride, TiCl4, is the compound which is released from the aircraft. It’s a volatile liquid which reacts immediately with the water in humid air to form thick clouds of ‘smoke’. The ‘smoke’ is actually a mixture of titanium dioxide, TiO2, and hydrochloric acid, HCl. These fumes don’t dissipate easily, remaining in the air so the white letters are visible against the blue sky. The reaction is as follows:
TiCl4 + 2H2O -> 4HCl + TiO2
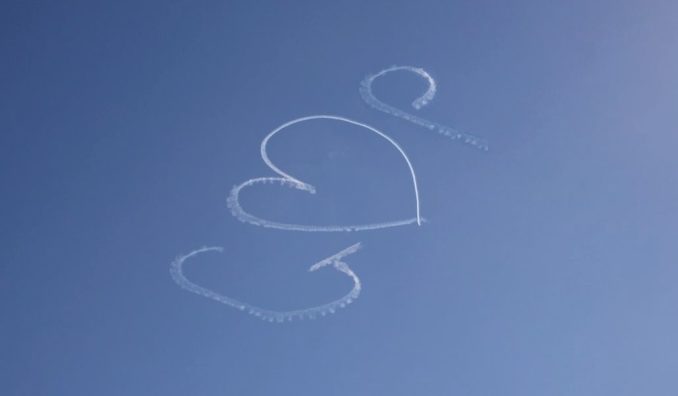
Adapted from Ethan Hartman, licensed under CC BY-SA 2.0
Incidentally, titanium tetrachloride, TiCl4, is a compound we met earlier in this piece. It is the compound which is produced in one of the steps to produce elemental titanium metal from its ores, the one which is often referred to as ‘tickle’.
Although most titanium compounds occur in the +4 oxidation state (since this is the most stable state), there are also numerous compounds in the +3 oxidation state.
Titanium(III) compounds, especially the halides, often exhibit a purply-violet colouration. One noteworthy example is titanium trichloride (TiCl3), titanium’s most common halide, which is a beautiful rich purple colour in solution. This compound is particularly important as it is used as a catalyst (it’s actually known as a Ziegler–Natta catalyst) in the production of polyolefin polymers. These make up common plastics such as polyethylene (a.k.a. polythene) and polypropylene. TiCl3 is a bit of a devil to handle, as it reacts readily with air and water, and can sometimes spontaneously combust. Another halide, titanium(III) bromide, TiBr3, can be used in a similar manner.
Titanium(III) oxide, Ti2O3, is a semiconductor. As Ti2O3 nanoparticles are conductive, it has found a use in forensic science. Though early days for its use yet, it has been found to help visualise latent fingerprints which cannot usually be seen by eye (these are often traces of sweat, oil, or other skin secretions) on a wide range of different types of surfaces. The tiny particles of Ti2O3 bind to the fingerprint residues, allowing them to be viewed (using optical and electrochemical imaging techniques) with high sensitivity, and resolution, then they can be compared to databanks of fingerprints to help solve crimes.
Titanium nitride, TiN, which is sometimes referred to as tinite, is an extremely hard compound (a ceramic) in which an atom of titanium is triple bonded to an atom of nitrogen.
TiN has found a number of uses. Its extreme hardness comes in handy in a number of applications. As well as the hardness, it has excellent resistance to corrosion, heat, and wear. It is used in crucibles for metal casting, to present a damage resistant surface in military and aerospace applications, and it is used in the production of medical tools, since it is also a biocompatible material.
Hence it finds a use in things like scalpel blades, bone saws, etc, helping to improve the service life of these instruments by a factor of three or more. In addition, as it is corrosion resistant, it is used as a coating on electrodes used in bioelectronic devices (e.g. medical implants or biosensors).
However, TiN also has lubricating properties as well as being wear resistant, which means it is ideal for coating surfaces, usually in tools, which need low friction to slide over one another.
Since it has a yellowish appearance, a layer of titanium nitride over another metal such as steel makes it look similar to gold at a much lower cost. Hence it can be used to coat items of jewellery to provide a decorative and hard wearing ‘gold-like’ appearance.
Whilst on the subject of personal adornment, titanium metal in jewellery has been around for a long time. I have a pair of earrings bought in the 1980s which exhibit glorious colours in the metal, and look as good now as the day they were purchased (at great expense) in the Burlington Arcade!
Normally, titanium is a silvery white metal, with a greyish hue. But, as for my forty-year-old earrings demonstrate, that colour can be modified by anodisation. This is a process in which titanium oxides are ‘grown’ onto an underlying titanium base metal using electrolysis. By varying the voltage applied, some gorgeous hues (e.g. pink, magenta, purple, blue, teal, green, gold, black, and rose gold) can be produced, adding to the beauty of the finished piece.
The metal is seeing a bit of a resurgence too and is becoming more and more trendy for jewellery making. For some time, it has been popular for men’s wedding rings, and also watches, since it is quite difficult to damage, though it isn’t invincible. But being lightweight and hypoallergenic, it is now becoming more fashionable for a variety of different jewellery, especially as its strength lends itself to the creation of delicate and intricate patterns.
Titanium is one of many other metals alloyed with gold (Au), to make it harder and more durable. Pure gold is referred to as 24-carat gold, but this is too soft to be used for jewellery, so it is almost always alloyed with stronger metals. We are used to seeing 9- or 18-carat gold jewellery, but if the alloy is gold and titanium, a tiny quantity, less than 1% of titanium is used to produce a durable item. Hence, the alloy is still classified as 24-carat gold.
But titanium has another thing of beauty to offer in jewellery terms, and to me this is of much greater interest. The attraction is due to a property called ‘asterism’, (from the Greek ἀστήρ which means ‘star’). In this optical phenomenon tiny needles of rutile inclusions (a.k.a. titanium dioxide, TiO2) are found in some precious stones, notably sapphires, but also in some rubies and, more rarely, topaz.
When cabochon stones (those shaped into a cushion-like stone and polished, not cut into facets) are viewed in direct light, the light is reflected by the rutile needles within the stone. It displays a six-rayed star appearing to float inside the stone, which shifts and sparkles as you tilt the piece.
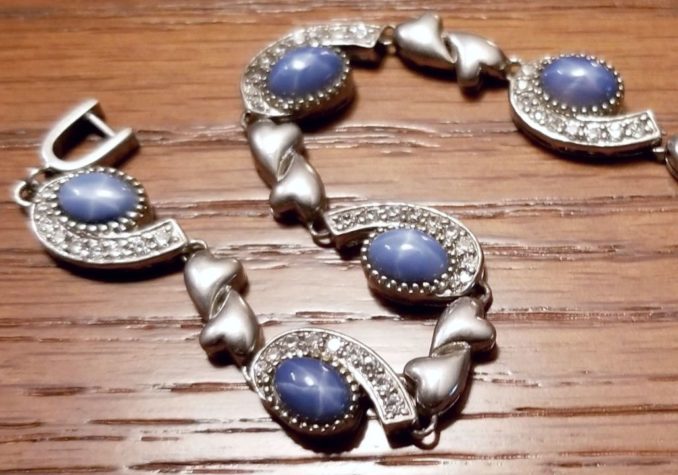
Terri Bateman, Public Domain
Although star sapphires are usually blue (as most sapphires are) the stones can actually vary in colour from a delicate pink, through orange, yellow, and green, to lavender (as seen above), even to grey or black. Usually, the ‘star’ appears to gleam white in appearance, but in some stones from Thailand the star is golden.
Needless to say, star stones have long been prized for their uncommon appearance. In the early years of the first century AD, Pliny the Elder described a stone from India “within which was the appearance of a star shining with the light of the moon”.
But they are also prized for their talismanic meaning, being seen as a lucky stone, particularly for travellers, and one which protects the owner, scaring away demons and warding off the evil eye. Even those who gaze upon their beauty were thought to benefit from its protection. In the past, these stones were referred to as the ‘stone of destiny’, as the three intersecting rays that formed the six-pointed star were linked to faith, hope, and destiny.
The 12th century Benedictine nun and abbess, Saint Hildegard of Bingen (also called the Sibyl of the Rhine), wrote a series of books known as the ‘Physica’, as a hands-on encyclopaedia of folk remedies and monastic medicine. She regarded sapphires as the most healing crystals for the soul. In more pragmatic terms, she believed that they could soothe painful eyes, ease migraines and neuralgia, and relieve insomnia. However, to star sapphires she attributed a great deal more, saying:
Adapted from sjwaddington, licensed under CC BY-SA 4.0
© SharpieType301 2023