By now, you should be getting quite used to meandering through the mesmerising memoirs of the elements. So far, we’ve covered Hydrogen, Carbon, Mercury, and Bromine. In doing so, we’ve also covered a good chunk of basic chemistry, and explored the development of the Periodic Table (a.k.a. the Periodic Table of the Elements).
With this element we’re on to round six. I’d like to say that this piece should be a little more relaxed. You may disagree, but I think it’s quite a gas.
I’m going to take a closer look at an element which is vital to our lives. One which is so essential to each of our body’s cells that without it, they will die within minutes. This time, our element is Oxygen.
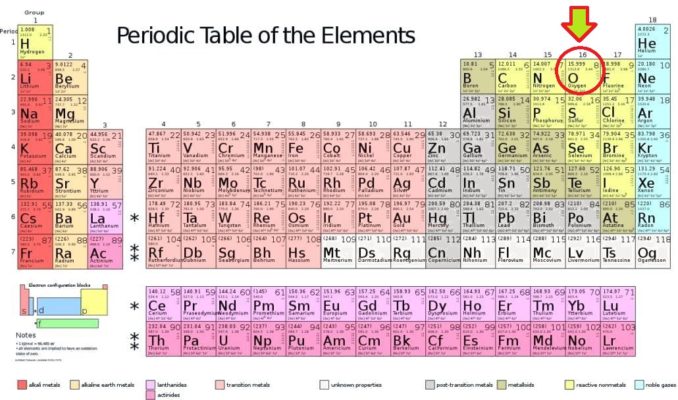
Modified from 2012rc, licensed under CC BY 3.0
As you’ll see from the Periodic Table above, oxygen lies in Group 16. This group is also known as the chalcogens. The name comes from the Greek ‘chalcos’ meaning ‘ore forming’, and the naturally occurring ones can all be found in copper ores.
Call me uncooperative, but I must admit that I find this group’s name a bit curious. Ores (minerals from which metals can be extracted) are generally found as oxides, and sulphides. That’s fair enough since sulphur, like oxygen, is one of this group’s elements. However, a considerable number of ores are metal silicates (in reality, the silicate minerals make up close to 90% of Earth’s crust). Hmmm, for me that accentuates the group’s name being a bit weird as silicon isn’t even found in this group, but in Group 14. Oh well, chemistry’s quirks strike again!
The elements of the chalcogen group are a bit of mixture. As well as oxygen (a non-metal), are other non-metals like sulphur (S), and selenium (Se). But there’s also the metalloid tellurium (Te), a semi-metal. Then there are two metals, polonium (Po), and the synthetic element livermorium (Lv), both of which are radioactive. Before we home in on oxygen, it’s quite fun to take a brief look at some of the other chalcogens.
Tellurium is one of the rarest solid stable elements found on Earth, although its abundance elsewhere in the Universe is rather higher. As a metalloid, it rather hedges its bets as its properties fall somewhere between those of a metal and those of a non-metal. It leans more towards a non-metal in the way it reacts chemically. It was first recognised as an element in 1783, isolated from gold-bearing ores mined in Zalatna, Transylvania, an important source of gold in Roman times. It’s occasionally seen in its native form, silver-grey crystals with a metallic lustre, which break along cleavage planes. It’s used in solar cells and semiconductors and is added to copper and stainless steel to form alloys which improves the ease with which they can be machined. Not a particularly pleasant element, it is slightly toxic, and has no known biological use. On exposure (especially to dust) victims develop characteristically smelly, garlicky breath, colloquially called ‘tellurium breath’. An interesting tale comes from the 1890s gold rush in Kalgoorlie, Western Australia. One of the main telluride ores, calaverite, had been overlooked as worthless pyrites or ‘fool’s gold’ and this was dumped as road fill on tracks and pavements around the town. Until someone caught on, the town’s streets had been, quite literally, paved with gold!
Polonium, again, is quite a noteworthy element, and an extremely dangerous one to boot. It was one of two radioactive elements discovered by Marie Curie (and husband, Pierre), and one for which she was awarded a Nobel Prize. It seems a little poignant that its name comes from Marie Skłodowska-Curie’s homeland, Poland, since the research which led to this discovery quite likely contributed to her demise, and possibly to that of her daughter Irene. I imagine that former Russian spy Alexander Litvinenko would rather that Marie had failed in her work, and polonium had not been isolated. It was this element introduced into a teapot of green tea which was used to assassinate him in London in 2006. Studies by the Swiss Institut de Radiophysique controversially suggested that polonium could also have been used to initiate the sudden decline and death of PLO leader, Yasser Arafat, a couple of years earlier. Equally chilling, brought together with beryllium, polonium was used as the modulated neutron initiator for ‘Little Boy’ and ‘Fat Man’, the atomic weapons dropped on the Japanese cities of Hiroshima and Nagasaki, on 6th and 9th August 1945, respectively.
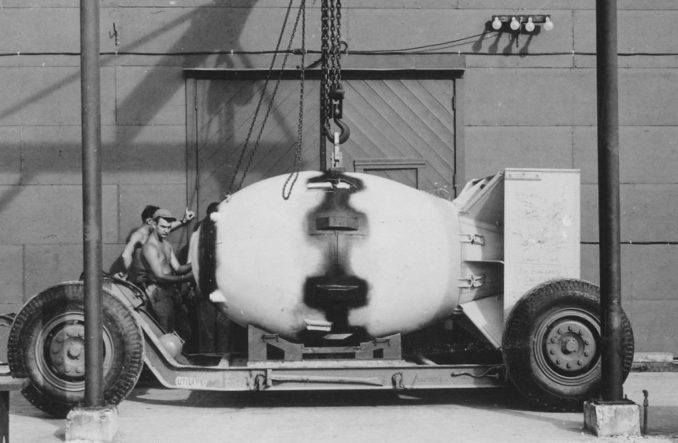
From Wikipedia, Public Domain
In contrast, the synthetic element Livermorium is, to my mind anyhow, not exactly thrilling. Whilst it’s expected that it ‘might’ share some similar properties with its lighter Group 16 cousins, no properties have yet been measured for this short-lived element. Perhaps I’m being a bit harsh, thinking it boring. I daresay my view won’t be shared with the Russian chemists who managed to synthesise this stuff in 2000.
Back to oxygen though, and our element actually is a little different in its properties to the other chalcogen elements. So, how is it different, and why?
To begin with, it exists as in the gaseous state, a colourless, and odourless gas which does not have an identifiable taste. This gas is present in (cue Sting) every breath you take. In our atmosphere, it is the second most abundant element, at c.21% (nitrogen gas is the most abundant, at c.78%). All the other elements in its group are solids.
The Group 16 elements have six electrons in their outermost shell—they are hexavalent, lacking two electrons to fill it. This makes them quite a reactive group, albeit a little less so than the halogens we looked at previously. That said, oxygen is much more reactive than the other chalcogens.
Why is this so? OK, I guess it’s time for a school chemistry alert! For those of you with a delicate constitution, feel free to skip a few paragraphs.
Firstly, as you look at the elements in turn across one of the periods (rows) in the periodic table, the atomic radius decreases (the atoms get smaller). This is because that there are increasing numbers of protons in the atom’s nucleus, and these are positively charged. The electrons in the outermost shell ‘feel’ the attraction of that positive charge from the nucleus more strongly. This pulls the electrons closer to the nucleus, so the radius of the atom is smaller.
Then, as you look at the elements in turn down one of the groups (columns) in the periodic table, the number of both protons and electrons increases (the atoms get bigger). With this increase, there are more electron shells. Hey, you might think, that means there’s more protons, lots of positive charge, so those electrons’ll get sucked in hard.
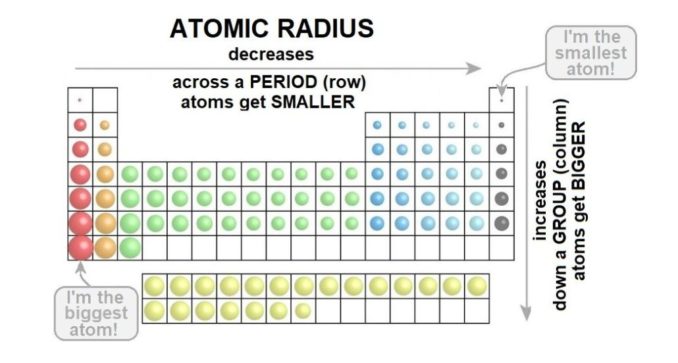
Adapted from Andrew Vreugdenhil and Kelly Wright, licensed under CC BY 4.0
Well, not quite. That’s because there is also an effect called electron shielding going on. While the positive protons in the nucleus attract the negative electrons, the electrons’ negative charges also repel one another a bit. In effect, the electrons in the inner shells ‘shield’ the outermost electrons from the attraction of the positively charged protons in the nucleus. Let’s have a look at this for the Group 16 elements.
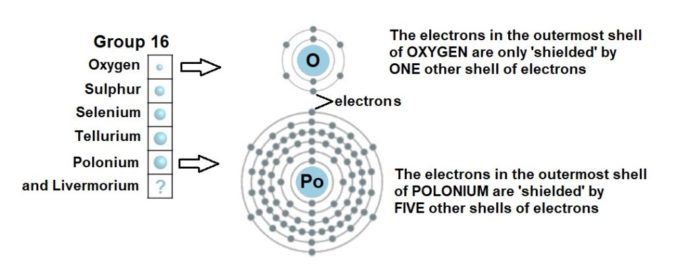
SharpieType301, 2023, adapted from Andrew Vreugdenhil and Kelly Wright, licensed under CC BY 4.0
The electrons in the outermost shell (especially in those heavier elements which have the largest number of electrons and electron shells) are subject to a sort of push-me-pull-you effect. This means those outermost electrons are not attracted so strongly towards the positively charged nucleus. This affects any reaction where bonds are concerned, because it’s the electrons in the outermost shell which are involved.
So, back to our oxygen, a small sized atom with only two electron shells to contend with. The negatively charged outermost electrons are pulled in tightly towards the positively charged protons in the nucleus. This gives oxygen the highest propensity in its group (indeed of any element except fluorine) to attract and pull in electrons to form a chemical bond. This means it is a very reactive element, and in chemistry terms, it has a high electronegativity and electron affinity.
Oxygen typically forms bonds by accepting (some might say stealing) two electrons from other atoms to complete its outer electron shell, though it can form bonds by accepting just one. This means that it will have an oxidation state of -2, or possibly -1 (remember, those electrons it accepts lead to a negative charge).
The other chalcogens differ though. To stabilise their outer shell, they can either accept electrons, or they can lose electrons (which aren’t so tightly held in place) to become more positive. Sulphur, for example, can have an oxidation state of -2, or -1 (by accepting electrons), alternatively +2, +4, or +6 (by losing electrons).
Unlike the other Group 16 elements, oxygen exists as a ‘dumbbell’-shaped diatomic molecule, O2, not as a single atom (in this it is similar to our previous element, bromine). This O2 is usually simply called oxygen gas, but is sometimes referred to as molecular oxygen, diatomic oxygen, dioxygen, or dioxidene.
So, who actually discovered oxygen? Well, the story still remains a little hazy to this day. In the late 15th century, as part of his careful studies of the human body, Leonardo da Vinci (perhaps the greatest polymath the world has ever known) was demonstrably interested in the lungs and how they functioned. He was quite aware that there was something in the air which we breathe that made it essential to life. What he didn’t accomplish was to identify this ‘something’.
Through the ages, alchemists recognised that both burning (combustion) and breathing (respiration) required air and conducted experiments to try to identify the ‘essence’ or ‘spirit of life’ which it must contain.
Then the Polish alchemist Michał Sędziwó, also known as Michael Sendivogius, did indeed isolate a substance from air which he termed ‘cibus vitae’, the aerial ‘food of life’, probably just prior to 1604.
This discovery possibly occurred while he was in Prague under the patronage of the reclusive Holy Roman Emperor, Rudolf II (the Alchemist’s Emperor). But it’s more likely that the discovery was made after he’d moved to Kraków. There, from around 1600, he conducted his experiments in chambers under Wawel Castle, at the court of King Sigismund III Vasa (another alchemy enthusiast).
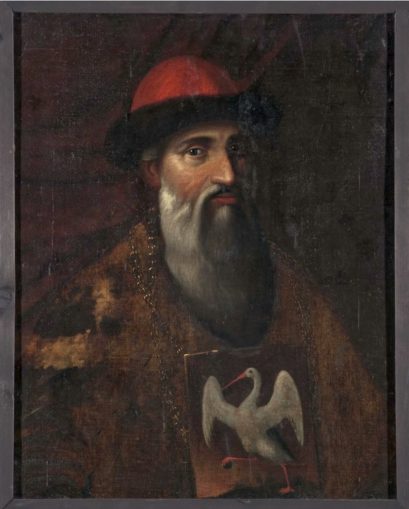
From Wikimedia, Public Domain
Sędziwój described the gas evolved when heating saltpetre in his manuscript De Lapide Philosophorum Tractatus duodecim e naturae fonte et manuali experientia depromti. However, his ideas were pooh-poohed as nonsense and charlatanism by alchemists and proto scientists for nearly two hundred years. It took until the late 18th century for the gas he described to be formally recognised as the element oxygen.
Thus, the credit for the discovery of oxygen usually goes to the British chemist Joseph Priestley. In 1774, he used a 12-inch-wide glass Tschirnhaus or ‘burning lens’ (which we’ve encountered in a previous article) to focus sunlight onto a piece of mercuric oxide sealed inside a glass container. The gas he collected, he then used in a series of experiments.
One of these involved placing a live mouse into the gas. The poor creature astonished him, as it didn’t keel over as expected. Indeed, it remained resolutely alive (though possibly not feeling too chipper) for around an hour, whereas he had anticipated mere minutes. This gas Priestley then decided was a particularly pure form of ‘everyday’ air saying that it was:
“five or six times better than common air for the purpose of respiration, inflammation, and, I believe, every other use of common atmospherical air.”
Great. He’d discovered oxygen, named it ‘dephlogisticated air’, and published his findings. This time the scientific world believed in his work. The only problem is that manuscripts from a Swedish chemist, Carl Wilhelm Scheele, indicate that he had produced the self-same gas by heating manganese dioxide with sulphuric acid as early as 1700, calling it ‘fire gas’. Unfortunately, foolish chap that he was, Scheele didn’t get around to publishing his findings until 1777.
But the plot thickens… both men, independently, were in correspondence with an old friend of ours, French scientist Antoine-Laurent de Lavoisier. His work allowed him to effectively debunk the long-standing ‘phlogiston’ theory. He’d both repeated some of Priestley and Scheele’s experiments and conducted his own, unequivocally demonstrating that air was a combination of substances not an element in its own right. By identifying the role this new substance (oxygen) played in atmospheric air, he proved that air comprised two components: one which supported and the other that opposed combustion and respiration. It was Lavoisier who called the gas oxygène, from the two Greek words ὀξύς (sharp, or acidic) and γενής (producer of). He might well have claimed credit for the discovery of oxygen, had he not met his rather sticky end at the hands of Mme Guillotine.
When we’ve explored other elements, we’ve looked at the element’s different isotopes and allotropes.
Oxygen has seventeen known isotopes, of which three are stable: 16O, 17O, and 18O. Of these, 16O is the most abundant (contributing about 99.7% of the oxygen found on Earth). Currently, unlike some of the other elements, oxygen’s radioisotopes haven’t found many uses.
That said, the radioisotope 15O is useful. Along with the stable isotope, 18O, it is used in isotopic labelling to enhance functional imaging techniques in medical applications. One such imaging technique is positron emission tomography (PET), which gives insight into the activity of cells by producing detailed 3D images of the inside of the body. PET scans can be used to track tumours and metastases, to look at heart function in detail, and to help diagnose types of dementia, e.g. differentiating Alzheimer’s disease from other causes of dementia.
By analysing the ratio of 18O/16O (δ18O) in geologic samples, palaeoclimate (ancient climates) can be reconstructed, providing insight into significant changes over time. Analysing δ18O has applications in archaeology too. Some human tissues are preserved better than others in an archaeological environment, meaning that they persist for us to study. These are typically bone, teeth, and sometimes hair (only rarely the squishy bits inside us). Analysing their δ18O, together with a good understanding of how isotopic ratios and signals pass from the environment (e.g. the water, and foods consumed by an individual) to be incorporated into body tissues, allows us to understand more about peoples’ diet, health status, and whether they have always lived in the same region. Fascinating to me, this topic would be an article all by itself!
Whilst we’re thinking about archaeology and oxygen, there’s a rather entertaining anecdote involving an ‘artefact’ (actually a hunk of pale blue, matte-surfaced ‘stone’) found in the Sierra Leone diamond fields. In 1990, controversial geologist (some say archaeologist) Angelo Pitoni purportedly obtained an odd stone from a tribal chief in Kono district. He dubbed this the ‘Stone of Heaven’.
So unusual was the stone that Pitoni had pieces chemically analysed at a number of labs around the world. They were found to be composed of c.77% oxygen, 20% carbon, with traces of carbon, silicon, calcium, and sodium. This quite unlike any known natural mineral, in fact closer, chemically, to a form of concrete or stucco. Interesting indeed, as the ‘stone’ is found in layers dating to c.12,000 BC, a time when most humans lived as hunter-gatherers.
The chief told Pitoni the story of the mysterious blue stones. According to legend, in ancient times the stones were angels. However, they were wicked, so God banished them from heaven. They fell to Earth, clutching a little piece of the sky and some stars, to be buried in the soil. This, the story goes, explains why this region is so rich in diamonds and minerals. Interestingly, the fourth largest diamond in the world, the Star of Sierra Leone (a 969.8 carat monster), came from Kono. Believe as you will, but it’s been suggested that the strange material must be the product of a highly advanced, lost civilization, perhaps even of extra-terrestrials. Weird, huh!
The other stable isotope, 17O, finds a use in nuclear magnetic resonance (NMR). This gives it a great number of applications in biology, including metabolic and biochemical studies. But as oxygen is such a reactive element, this isotope also allows a raft of other applications, to study both organic and inorganic compounds, including metallic compounds.
As to oxygen’s allotropes, well, the one we are most familiar with is molecular or diatomic oxygen, O2. This is the stuff we breathe in. It’s also known as dioxygen or triplet oxygen, and it is the most stable allotrope.
When it cools sufficiently to form the icy-blue liquid oxygen (which you’ll know as LOx), it is used in industry, medicine, and scientific research and, notably, in the aerospace industry as a rocket propellant.
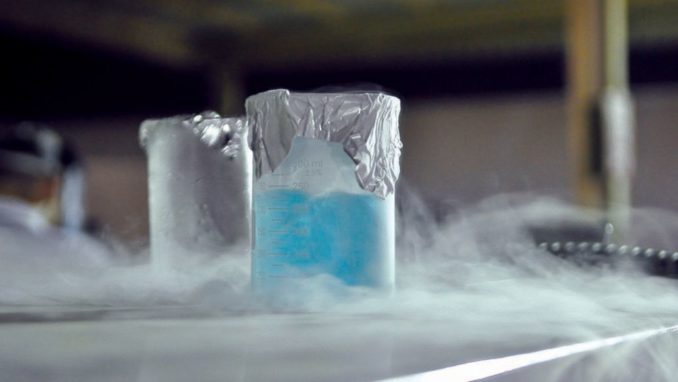
From Wikimedia, Public Domain
You will almost certainly also be familiar with another allotrope, ozone. This is also a gas, this time a pale blue gas (though dark blue in liquid form, and purple-black as a solid). It’s ozone that gives the characteristic sharp smell after a bolt of lightning or electrical discharges. Ozone, O3, is pretty unstable and is an incredibly powerful oxidising agent (electron acceptor)—much stronger than O2. This makes it extremely reactive, and also potentially hazardous. However, ozone has a number of practical applications, including therapeutically, of which we’ll hear more shortly.
There are a couple of other allotropes of interest. The first, O4, tetraoxygen (also called oxozone) is not naturally occurring but has been shown to have some potential applications, specifically in rocket propellants (for oxidising rocket fuel) instead of LOx. It has been produced in a stable, closed ring form, as a non-toxic liquid which is safe to handle at room temperature. This is a real bonus since LOx (liquid oxygen, which is more commonly used), as you’ll see in the photo above, boils unless kept under cryogenic conditions, thus readily evaporates into a rolling cloud of cold gas. More problematic though is that, even at extremely low temperatures, LOx is extremely explosive once it comes into contact with rocket fuel. Tetraoxygen is a bit easier to deal with.
The other allotrope, O8, is solid oxygen. This exists at temperatures below −218.79 °C (54.36 K). Depending on the pressure, it can form in six different solid phases (with distinct crystal structures). Interestingly, it takes on quite different colours according to its phase, from a pale cerulean-blue, to pink (with a hint of pale blue), colourless with a faint blue tinge, orange, red or a dark reddish-black, and ‘metallic’-black. Each phase has distinct thermal, magnetic, and optical properties. This makes them potentially useful, so they (especially the dark red epsilon ε-phase, which is particularly unusual) are certainly being investigated by researchers, although specific practical applications have yet to be established.
From here onwards, most of this episode will focus on molecular oxygen. In the air we breathe, oxygen typically makes up around a fifth (c. 20.9%). The remainder of air is predominantly nitrogen. Water vapour (the amount varies depending on where you are and from season to season), argon, and carbon dioxide are the next most abundant elements. Between them, these five account for around 99% of the constituents of air.
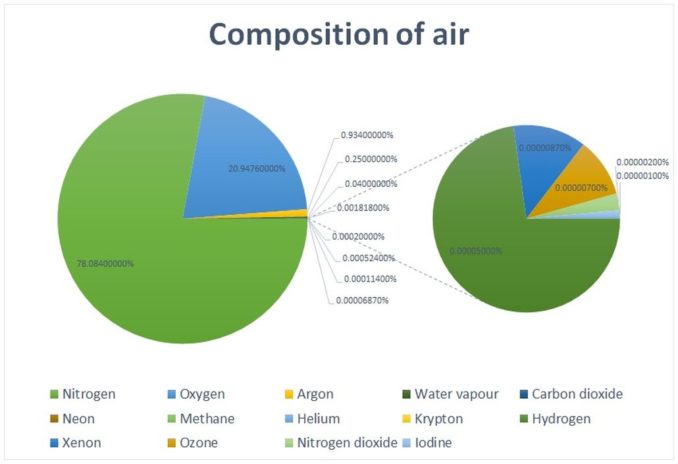
SharpieType301, 2023
Oxygen is vital to sustaining life. We’ll take a look at how in a little while, but it is interesting to know that, for our purposes, the precise percentage of oxygen in the air we inhale is critical.
Air monitors used in laboratories and industry typically set off an alarm when oxygen levels drop to 19.5%. At this percentage air is judged to be oxygen deficient. Conversely, oxygen levels in excess of that typical 21% are considered to be oxygen enriched. This is a decidedly narrow band.
Why is this important to us? Well, without sufficient oxygen, our body’s cells are rendered incapable of producing the energy they need to survive, so they die. This can begin to happen very quickly, within just minutes.
Low levels of oxygen, hypoxia as you may have heard it called, damages the brain (this organ demands around 20% of all the body’s oxygen). At its worst, say when blood flow and the oxygen it carries to the brain is obstructed, brain cells will die, and an individual will suffer a stroke. This is a major cause of disability and death.
Even when the oxygen in the air we breathe drops by just a few percent there is an impact. At 19% there will already be some physiological effects, but as it drops to 16% pulse rate increases, breathing becomes more rapid, and people exhibit impaired thought processes, poor coordination, and an inability to focus and concentrate.
By 12.5%, breathing is more severely affected, an individual may become nauseous or vomit, and there’s now a risk of permanent heart damage. Drop the level to 10% or less, and convulsions are likely. An affected individual may be unable to move, lose consciousness and possibly even their life.
The body can tolerate a little upward deviation from the air’s typical 21% as oxygen levels rise, but too much (hyperoxia) and oxygen becomes toxic. While oxygen toxicity is unlikely to be a problem in everyday life, it becomes a danger for divers, and for anyone being treated with high concentrations of supplemental oxygen (e.g. patients on ventilators, premature babies, or those needing hyperbaric oxygen therapy). The effects can be life-changing and permanent.
Hyperoxia occurs when oxygen levels are higher than normal, and primarily affects the central nervous system, lungs, and eyes. That said, oxidative damage can affect any cell in the body so can be more insidious, destroying the body’s DNA, and damaging the proteins, carbohydrates, and lipids that our body needs to function normally.
Oxygen toxicity’s effect on the lungs, actually the first part of the body to be damaged, results in chest burning or pain, difficulty in breathing and uncontrollable coughing. If it continues, the alveoli (the little air sacs where gas exchange takes place) can collapse.
The impact on the central nervous system is severe. The initial signs may appear relatively mild, but they are anything but. Tunnel vision, ringing in the ears, and facial muscle twitches, are experienced at the start. Loss of coordination, headaches, dizziness, and behavioural changes (someone becoming confused, irritable, or anxious) may follow, but symptoms worsen over time. Unless the oxygen levels reduce, convulsions follow—grand mal-type seizures with classic tonic–clonic phases, leading to a loss of consciousness, a particular problem for divers as this poses a serious risk of drowning.
In the eye, the delicate retina (the light-sensitive layer which allows us to see) is affected by prolonged exposure to elevated oxygen levels. Initial blurred vision can, with continued exposure to elevated oxygen levels, lead to short-sightedness, the development of cataracts, or to partial or complete retinal detachment.
Glam rockers Sweet summed up this fine balance (er, sweet spot?) pretty well, comparing love to oxygen in their 1978 Top Ten hit:
Love is like oxygen,
You get too much, you get too high,
Not enough and you’re gonna die…
OK, we’ve seen what too much or too little can do to us, but why is oxygen such a critical element for us, as humans?
Well, to start with, it is actually the most abundant element in the human body. There’s more oxygen in our makeup than there is carbon, a rather interesting concept for a ‘carbon-based’ life form, which I guess gives us some idea of its importance to us. It comprises some 65% of what is ‘us’. It’s in body water (of which we are c.55-60%), and it’s in the carbohydrates, lipids, nucleic acids, and proteins which form us.
There are actually eleven elements which are fundamental to life. The ‘big four’ (oxygen, carbon, hydrogen, and nitrogen), and a further seven which play a major role, as shown below. About 99% of the mass of the human body is made up of the first six of these. However, trace amounts of a further fourteen elements (boron, chromium, cobalt, copper, fluorine, iodine, iron, manganese, molybdenum, selenium, silicon, tin vanadium, and zinc) play an important role in keeping us healthy.
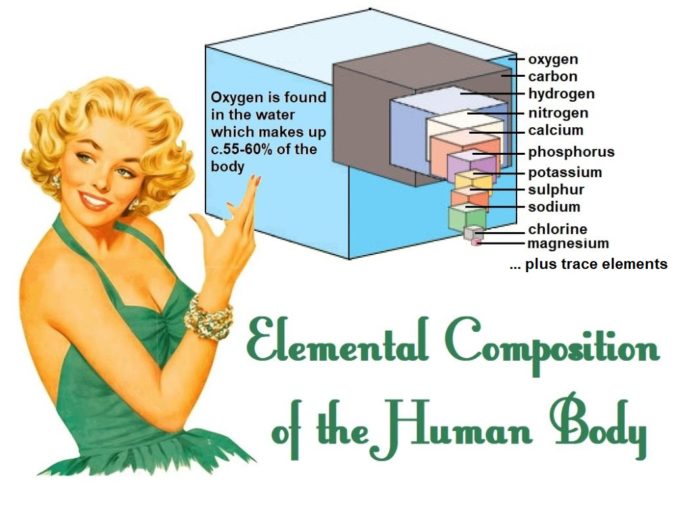
SharpieType301, 2023, adapted from Karen Arnold, licensed under CC0
Whilst you are sitting quietly, casting your eyes over these words, and breathing, there is a lot going on inside your body. Hundreds of thousands of biochemical reactions are taking place every second at the cellular level, and this goes on every second you are alive. These reactions are essential for life and many, if not most, rely on oxygen. If you are interested in how oxygen is taken up from the air to move around our body, there’s a fascinating TED-Ed talk about Oxygen’s surprisingly complex journey through your body.
This wealth of biochemical reactions is all part of metabolism, a complex biological ‘dance’ which allows your body to create, maintain, and repair cells, and dispose of damaged tissues, recycling as much as possible for use in other body functions. It helps you digest the food you eat, manage waste products, balance body chemicals and hormones, and regulate your body temperature. It’s needed for your heart to continue to beat, your blood to circulate, for your brain and nerves to process and transmit information, and your muscles to function thus allowing movement, including breathing. It’s essential to your immune system, for reproduction, and much, much more. Every single one of these basic body functions requires energy.
Inside all of our cells, except our red blood cells, are tiny organelles (mini ‘organs’, if you like) called mitochondria. These small, specialised structures are sometimes referred to as the powerhouse of the cell. Their main, though not sole role is as energy generators—little biological power plants. They use the oxygen we breathe in (molecular oxygen, O2) to transform the food we eat into energy that can be used by our body. Oxygen does this as an electron acceptor, taking us right back to where we looked at chemical bonding earlier in this piece. The compound which drives this process is adenosine triphosphate (ATP), sort of molecular ‘cash’ used in the transactions of life, and its story is definitely worthy of an article of its own someday. Since we are now straying into the world of biology, I’ll leave it here.
Let’s take a look at some non-biological uses of our element. We’ve already seen that liquid oxygen (LOx) is used as part of the fuel for rockets. But there are many more common industries which make great use of this element for a variety of purposes. Oxygen is a commonly used gas in the manufacturing of semiconductors, in part when the silicon layers are deposited, but also as a high-purity gas to drive off contaminants and by-products that can affect the quality of the finished semiconductors.
As chlorine is gradually phased out due to environmental concerns around water pollution, oxygen is now being used more and more as an important bleaching chemical in commercial papermaking. Papermaking relies on a complex range of chemical and mechanical processes. To produce a high-quality, white paper, the lignin in the wood pulp must first be removed in a ‘bleaching’ process, to leave the cellulose fibres behind. Using oxygen and caustic soda (sodium hydroxide) in this step, rather than hypochlorite and chlorine dioxide, reduces manufacturing costs but there are other benefits to this process which are said to be helping make papermaking leaner and greener.
Oxygen has a great many uses as a raw material in the chemical industry, and also to produce chemical feedstocks or as a precursor for other chemical processes. For example, oxygen is used to produce ethylene oxide, a chemical which is then used in the production of a variety of products, from detergents to antifreeze, textiles to pharmaceuticals. It’s also used to produce propylene oxide, a precursor to polyurethane plastics, the most commonly used plastics for injection and cast moulding.
In petrochemical plants and refineries, oxygen is used to regenerate the catalysts used in the ‘cracking’ process (which converts heavy petroleum, crude oil, into lighter, more usable hydrocarbon products). This extends their lifespan, greatly reducing costs and increasing output. In addition, many crude oils and gas reserves (notably ‘sour’ crudes and gases) are rich in hydrogen sulphide (H2S). Both refineries and natural gas processors need to ‘sweeten’ their products by removing this contaminant, to comply with increasingly strict environmental regulations with respect to air pollution. For many years, the industries have used the Claus sulphur recovery process (SCOT) to accomplish this. More recently, it was realised that it is possible to recover elemental sulphur more efficiently from the hydrogen sulphide by enriching the airflow with oxygen. What’s more, using this method is nicely cost-effective.
The largest industrial users of oxygen can be found in metal manufacturing, both ferrous and non-ferrous. The steel industry is foremost amongst these, though large volumes of oxygen are also used in producing copper, lead, and zinc. Making steel relies on high temperatures, and one way to achieve these is to add oxygen to the air or other gases used in blast and other furnaces. An additional benefit to using oxygen is that the amount of fuel used can be reduced. Using the Linz-Donawitz process, the basic oxygen steelmaking process, means that furnaces can convert c. 350 tons of iron into steel in less than 40 minutes. In an open-hearth furnace, this would take some 10–12 hours.
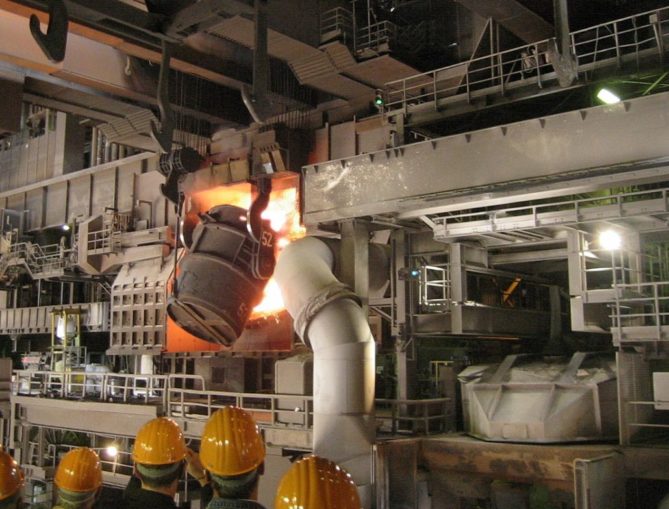
Katpatuka, licensed under CC BY-SA 3.0
Furthermore, the oxygen process means that noxious emissions (notably carbon dioxide) can be decreased. For similar fuel-saving and emission-reduction reasons, both glassmaking and the ceramics industry also make good use of oxygen. Once a metal has been produced, oxygen is also used in finishing processes, for example in oxy-acetylene welding and for cutting metals. For example, in the automotive industry, oxygen is used as a plasma cutting gas.
The fact that enhanced combustion temperatures can be achieved by enriching air with oxygen can also help with the management of hazardous waste. This is greatly beneficial since some waste is a quite a challenge to deal with. This includes clinical and medical waste, which can be infectious or radioactive, laboratory waste (some of which may be unidentified), prohibited drugs and other pharmaceutical products, pesticides and, believe it or not, commercial and household paints. But, with the extremely high temperatures in incinerators, commonly reaching 1,200–1,600°C, toxic compounds are broken down and, to all intents and purposes, thoroughly destroyed. There are some types of toxic waste which can only be safely destroyed by this method. These include cyanide-based compounds, chlorofluorocarbons (CFCs, once used as refrigerants), and polychlorinated biphenyls (PCBs, once used in electrical equipment, plastics, and pigments). Some modern High-Temperature Incinerators (HTIs) are even able to deal with certain radioactive waste materials. High-temperature incineration may entail the complete combustion of materials, or the hazardous material can be converted into usable, non-toxic substances. Better yet, it is possible to reclaim some of the energy used in this process by reusing the gas and heat produced by incineration, and even flue ash can be used in road-fill or producing concrete bricks for construction.
Oxygen (sometimes in the form of in the form of ozone, O3) has also found a use in wastewater treatment to ensure that water-based effluent, whether from domestic or industrial use, is safe for discharge into the environment. Treating sludge or sewage with oxygen, rather than only the traditional aeration methods, helps manage the organic matter contained in untreated water which can otherwise have a negative impact on aquatic organisms (both fauna and flora). Oxygen is forced through the water (or sludge) to increase the production of bacteria which break down organic wastes. Again, there are energy saving, hence cost implications in this, and it is a process which doesn’t require large-scale changes in existing plant, so can be used flexibly, on an ‘as required’ basis. An additional benefit is that the offensive smells (e.g. hydrogen sulphide, and volatile organic compounds) associated with treating wastewater are reduced.
When it comes to unwanted smells, oxygen in the form of ozone is also used to eliminate odours from tobacco smoke, pets, decaying food, mould and mildew, and other organic nasties in carpets and upholstery. Ozone provides an alternative to chlorine for use in commercial and institutional laundries too. As it’s effective in short washes at cold temperatures, its use can reduce energy bills as well as the cost of treating wastewater from the process. It disinfects as well as cleaning clothes.
Ozone can rapidly destroy bacteria, viruses, fungi, and algae, so works well in many applications as an environmentally friendly biocide and sanitiser. It leaves no residue or problematic by-products and since it can be used both as a gas and in aqueous form, it’s possible to sanitise surfaces that can be difficult to access, for example grain in silos. It can be used to control biofilms that build up in cooling towers, and is used in heating, ventilation, and air conditioning (HVAC) systems.
In a less well-known application, injecting pure oxygen (or ozone) into the water in which fish are farmed allows a higher weight of fish to be raised (or transported) in a given volume of water. It helps accelerate their growth by boosting food conversion and lessens the risk of disease. Similarly, modifying the atmosphere in which fresh produce is stored allows for a longer shelf-life, and larger transport boundaries, and minimises degradation. Oxygen, along with other gases, is used for this.
I will leave you with a magnificent aspect of oxygen, one of which until writing this article I was completely unaware. These are the aurora (in northern latitudes, the aurora borealis or the northern lights), those fabulously shifting, swirling displays of brilliant colours which can appear as flickers, arcs, rays, or nebulous curtains of light swathing the night sky.
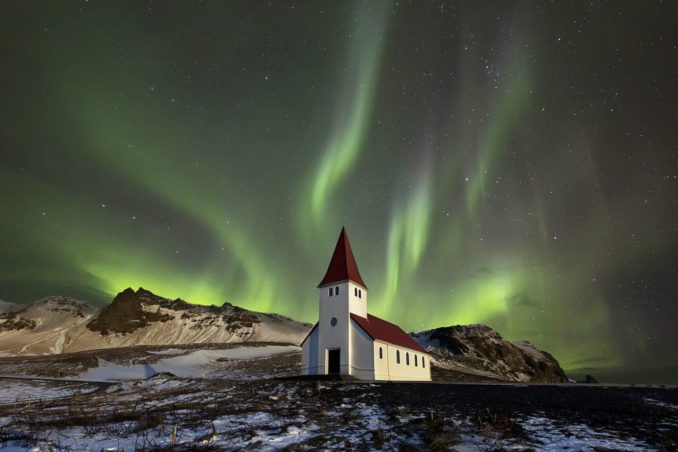
AstroAnthony, licensed under CC BY 4.0
The glorious lights you see is caused by charged solar wind particles colliding with oxygen atoms in the ionosphere (the ionised region of Earth’s upper atmosphere), ionising the atoms. When the ions return to their ground state, energy is released as light. The colour of light emitted is unique to the element and for oxygen the colours are typically green to greenish yellow, and red. Other colours such as blues and purples are the result of other ionised atoms (often nitrogen), but colours can also be modified by scattered sun or moonlight. Very occasionally, the lights can be accompanied by their own eerie music, a series of faint pops, hisses, and crackling, like radio static.
For the best chance to see this fabulous phenomenon, you ideally need to be within the ‘auroral zone’, typically a narrow band (around c.3°-6° wide) between 10° and 20° from the Earth’s geomagnetic poles. Realistically, that falls in areas around the Arctic. Now and again, when the circumstances are just right, for example when there is a geomagnetic storm, it is possible to see the aurora at latitudes outside of the auroral zone, but you really do have to be fortunate. One day, I hope to be in the right spot at the right time.
© SharpieType301 2023